Manufacturing of Glass
MANUFACTURING OF FLAT GLASS
Flat glass manufacturing is a key industrial process and has been subject to continuous development to achieve lower cost and higher quality since its transition from the craft of mouth-blown glass. Float glass is the dominant process for flat glass, while rolled plate remains in use for patterned (textured) glass and wired glass.
Glassmaking Materials
The key constituent is sand, which provides the silica (SiO2) matrix, and glass making historically evolved in locations with a source of pure silica sand. Pure silica melts sharply at about 1600°C and forms a dense glass with high refractive index on cooling. On slow cooling in nature, silica forms crystals of quartz or coloured gem stones like amethyst, ruby and sapphire according to the presence of small amounts of impurities (elements other than silicon).
The high melting temperature and narrow temperature range over which the material can be formed make pure silica glasses impractical for most purposes. The process of making glass in craft or industry involves the addition of ‘fluxes’, which are other minerals that lower the melting point and widen the range of workability.
Adding soda (Na2O) in the form of soda ash (Na2CO3) lowers the melting point by about 500°C but leaves the glass soluble in water. Adding lime (CaO) to the soda glass, in the form of limestone, calcium carbonate (CaCO3) makes the glass insoluble and widens its working range. Cullet (broken glass) forms a key part of the batch, improving heat transfer during melting and acting as a flux. Soda lime glass produced industrially also contains dolomite, which adds some magnesium oxide (MgO), and a number of other metal oxides in small quantities to control the melting point, working range and colour.
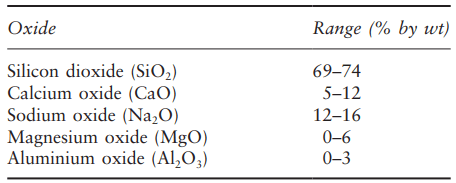
The range of float glass composition is shown in Table 1, but each float plant tends to be optimised to a different mix to take advantage of the locally available minerals and the design of the equipment, so chemical analysis can sometimes be used to trace a sample of float glass to a particular plant or manufacturer.
Composition
Iron oxide in the raw materials gives the glass a light green colour, which has become standard in Europe, but commonly produced glass in other parts of the world has a variety of tones. There are several ‘low iron’ glasses available that have a much whiter appearance. Glass used in buildings is almost exclusively soda lime silicate glass, which is defined by a series of European standards in its basic form and when processed in a variety of ways (BS EN 572 and BS 952).1
Borosilicate glass is also produced industrially by the sheet and float processes, and is used principally for its fire resistance because it has a lower coefficient of thermal expansion, which means it is less likely to crack when heated rapidly in a fire. It is also very widely used in tube form for handling chemicals, for instance as pipe work in laboratories and chemical plant. The chemical composition of borosilicate glass is approximately 70% silica, 10% boric oxide, 8% sodium oxide, 8% potassium oxide, and 1% calcium oxide.
Constituents and Microstructure of Glass
The microstructure of glass is dominated by the nature and arrangement of the bonds between the silicon and oxygen atoms that form the silica matrix. The fundamental characteristic of a glass is that it has an amorphous microstructure, meaning no shape or order, so there are no crystalline regions, as illustrated in Fig. 1.
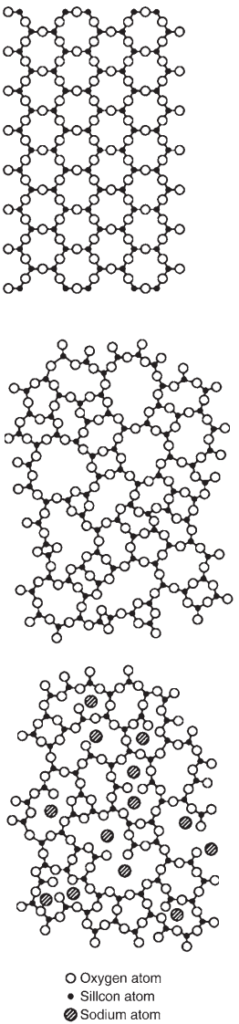
The significant thing about an amorphous structure is that there are no slip planes, dislocations or grain boundaries to enable plastic flow and impart toughness. Once a crack has been created in a glass, it will encounter no change in properties as it progresses through the material, so it can grow deeper and become a more intense stress raiser until it causes fracture or the load is relieved.
Historical Processes
Flat glass making started as a craft process using simple tools and developed into the highly mechanised industry of today through several steps. Crown glass was produced by glass blowing techniques and, with considerable skill, a ‘crown’ of up to 1.1 m diameter could be spun. The surfaces were smooth and fire polished but the thickness varied and circumferential lines were common. Each crown would be cut into rectangular sizes and the ‘bull’s eye’ at the centre was the least valuable, only used where necessary in cottage windows.
Cylinder glass also started by blowing but the bulb was swung on the end of the blowpipe to elongate it as far as possible. The resulting cylinder had its ends cut off and was split lengthwise to open out and anneal on an iron slab. Larger sizes could be made by this process once it became mechanically assisted, but the surface quality was not so good because the glass lay in contact with the iron slab while hot.
Sheet glass is drawn up from a tank through a slot in a ceramic die and gripped by toothed rollers at the edges of the ribbon. Drawn sheet glass has very smooth, fire polished surfaces, tends to have continuous ‘draw lines’ and is still commonly seen in houses built in the 1930s.
Rolled Glass (including wired and polished wired)
Patterned glass and low optical quality glass for horticultural use is produced by the rolled plate process, in which the molten glass is poured between a pair of temperature-controlled iron rollers. The ribbon is transported horizontally on ceramic rollers as it stiffens and cools until annealed and cut automatically. The lower iron roller is engraved with a pattern to create the texture in the glass, which may be for decorative or obscuring purposes (Fig. 2 top).
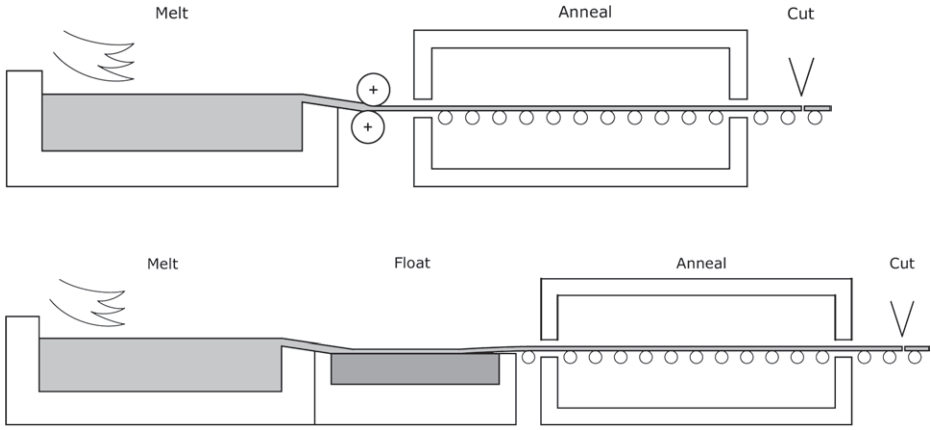
Wired glass is produced by the rolling process, but with the flow of molten glass divided into two streams, one above the other, two ribbons of hot glass are brought together continuously with wire mesh fed between the two. Further rolling joins the ribbons of glass around the wire and forms textured surfaces to the finished glass. If wired glass with smooth surfaces is required it is mechanically polished to remove the surface texture.
Wired glass was the first kind of glass to have any structural integrity after breakage, so it was often used for overhead applications before the introduction of laminated glass, but it does not have the toughness to be an effective safety glass and it is weaker than float glass because of the internal defects caused by the encapsulated wire. Wired glass can provide 30 minutes integrity against fire when properly framed, and is often used for this property in doors and small windows.
Float Glass
The float process provides over 90% of flat glass production, and has been dominant since its rapid licensing to all the major glass manufacturers by Pilkington during the 1970s, following its development in the early 1960s. In a continuous process, glass is melted from the mineral batch, refined in a tank where the molten glass moves slowly to allow bubbles to escape, then flows onto a bath of molten tin, where it floats and naturally forms a ‘ribbon’ about 6 mm thick. The interface between the glass and the molten tin is perfectly flat and the top surface is smooth and ‘fire polished’.
As the ribbon is drawn across the tin bath it is allowed to cool from about 1100°C to around 600°C, at which temperature it is rigid, and it progresses onto a series of ceramic rollers. The rollers carry the continuous ribbon through the ‘lehr’, a continuous oven in which the temperature of the glass is lowered gradually and evenly so that it emerges in the ‘annealed’ condition, i.e. with very low residual stress (Fig. 2 bottom).
The cutting of float glass is fully automated and the glass is stacked by robots into packs of typically 2 tonnes for distribution in loads of 20 t. The standard maximum size in Europe is 6000 mm by 3210 mm, and is known as a ‘jumbo sheet’. Longer ‘super jumbos’ up to 8 or 9 m long are available from some float lines to special order, and a very few lines can produce extremely long sheets up to around 12 m, but elaborate handling equipment has to be arranged to off-load and transport such glass.
A typical float line produces around 500 t of glass per day and can be adjusted to produce a range of thickness. The thinner ‘substances’ have to be stretched out as they cross the float tank, and to make heavy glass the ribbon has to be constrained to build up the thickness. Changing from one thickness to another takes time as the glass flows continuously, and the process is allowed to settle within the tolerances of the next thickness. Float lines operate a system of ‘campaigns’, in which the substance is stepped up progressively to the maximum and then down to the minimum, with a planned volume of production at each thickness. Float glass for buildings is available in standard thicknesses (defined in BS EN 572-2) of 2, 3, 4, 5, 6, 8, 10, 12, 15, 19 and 25 mm.
Some float lines specialise in body tinted (coloured) solar control glass, where additional minerals are added to pigment the glass to absorb more infrared energy and visible light. Body tints in green, grey, bronze, blue and pink are available in a limited range of thicknesses. It can take a matter of days for a float line to change from one colour to another, as the old composition flows out of the tank and the new composition flows through consistently, and the thickness and quality settle down.
COATINGS
Float glass is commonly coated to alter its transmission of energy or other surface properties. Some coatings can be applied on the float line while the glass is hot, by the process of chemical vapour deposition, which is highly economical. These ‘pyrolitic’ (high temperature), on-line coatings are hard, strong and durable because they are intimately joined to the glass surface. Other coatings, known as ‘off line’ or ‘soft’ are applied by the separate process of magnetron sputtering at room temperature but under high vacuum.
The glass enters a continuous series of vacuum chambers, in which the top surface of the glass is exposed to an electric plasma that bounces metal atoms off a solid metal ‘target’ to deposit as a layer on the glass. Traces of gas can be introduced to the plasma so that the deposited layer is a pure metal, an oxide or a nitride. Pure silver layers are used as part of a ‘stack’ of up to 12 coatings designed to work in combination to achieve the desired spectral properties.
Low-emissivity Glass
Glass naturally has an emissivity of about 0.9 to the broad spectrum of infrared energy emitted around room temperature. Since emissivity and absorbtivity are two expressions of the same physical property, this means that glass will absorb 90% of heat energy that is radiated to it from the people and interior of a room, and will radiate it equally readily across the insulating cavity of a plain double-glazed unit. To improve the insulating performance of double glazing, low emissivity – ‘low-e’ – coatings are applied very widely by on-line (pyrolitic) and off-line (sputtering) processes.
Solar-control Glass
Direct solar energy consists of a few percent ultraviolet, about 47% visible light and about 50% short wave infrared radiation, which passes easily through the glass to the interior. In cold climates and green houses this ‘solar gain’ is beneficial and can be retained by the use of ‘low-e’ coatings (see above) but in hot climates, or buildings with an excess of internal heat gains and large glass areas, the solar gain can place a large load on cooling systems.
Solar-control coatings are designed to reflect the short wave ‘near infrared’ radiation back into the environment and allow visible light through to the interior. The best modern coatings have very little effect on the balance of visible wavelengths and so the light retains it natural colour, which is measured as the ‘colour rendering index’.
Selective, High-performance Glass
Late 20th, early 21st century architecture pursued the ideal of a comfortable but transparent clear glass building with an invisible barrier between inside and outside. A range of clear solar-control coatings with very high light transmission, good colour rendering and low emissivity to give good thermal insulation, were developed. They are known as ‘high performance’ or ‘highly selective’ because they select between the visible light to be transmitted and the infrared to be reflected outwards or inwards.
Self-cleaning Glass
There are two competing strategies for making glass ‘self cleaning’ or ‘reduced cleaning’. The first products were liquid applied coatings offered as after-market treatments, which make the glass water repellent or ‘hydrophobic’. The objective is that water does not wet the glass surface but forms beads that run off easily, carrying away dust particles.
The effect is similar to that of the surface of a well polished car, a lotus flower or a fresh cabbage leaf. The latest generation of self-cleaning coatings are based on the ‘super-hydrophilic strategy’, in which the glass surface is made highly attractive to water, so that surface tension is overcome and the water wets the surface thoroughly. The coating of titanium dioxide is applied on the float line, so it is an integral part of the surface and highly durable. The coating acts as a catalyst under ultraviolet light, which breaks down organic deposits assisting the self cleaning action.
STRENGTHENING PROCESSES
Toughening (Tempering) with Heat Soak Test
Thermal toughening, known as tempering in North America, is based on the phenomenon that glass contracts further at high temperatures if it is cooled more slowly. The technique is to raise the temperature of the glass evenly above its transition point, say to about 620°C, when it is starting to become soft, and then cool it evenly on both surfaces (Fig. 3).
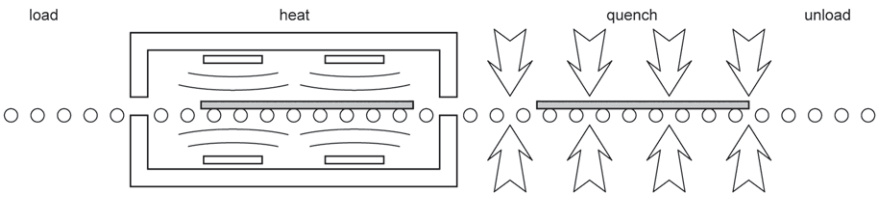
The surfaces harden quickly and the core of the glass sheet contracts as it cools more slowly, producing a balanced distribution of tension in the centre of the sheet and compression at the surfaces. Typically the surface compression would be around 100 MPa and the central tension about half this value, with the distribution being parabolic through the thickness (Fig. 4).
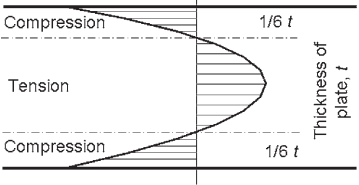
toughened glass.
With the surface of the glass in a state of permanent compression, any applied stresses must overcome the residual stress before opening surface flaws that could cause fracture. The core of the glass is generally free from defects and so is able to resist the residual tension permanently.
Toughened glass can be broken by penetrating the compressive layer at its surface so that a crack enters a zone where the residual stress is tensile. This can be caused by hard impact or by sustained contact with hard materials, especially where sharp features concentrate the contact stress.
The residual compression stress effectively increases the strength by a factor of about four and provides a proportion of strength that is unaffected by the duration of loading. Secondly, when broken, the stored strain energy drives a process of crack branching that spreads throughout the pane in a fraction of a second and divides it into roughly cubic fragments. This characteristic fracture pattern is much less prone to cause cutting and piercing injuries and for this reason properly toughened glass can be classified as a ‘safety glass’.
Its first widespread use was in car windscreens, and it is still used for side windows and rear screens but has been replaced in windscreens by laminated glass. Toughened glass is only able to sustain the high residual tensile stress that exists at its core because there are no flaws or cracks in that region to weaken it.
However, certain types of inclusion, notably those of nickel sulphide (NiS), undergo a solid-state phase transformation that can cause cracks that lead to what is known as ‘spontaneous breakage’. Nickelbearing ores and metallic nickel, such as is found in stainless steel, are eliminated from the whole process of mining, transporting and preparing the batch for glass making as far as possible, but occasional contamination is inevitable in processes consuming hundreds of tonnes of raw material per day.
Nickel in the melt reacts with sulphur, which is a common contaminant, and tiny globules of nickel sulphide form in the glass. At the high temperature of the float tank, nickel sulphide adopts a close-packed crystal structure know as the ‘alpha phase’ but at room temperature its stable ‘beta’ phase is a less dense structure, so nickel sulphide inclusions tend to expand over time as they transform.
In annealed glass this is not a problem but when this happens in toughened glass it can cause a tiny crack that initiates fracture. Nickel sulphide inclusions are only critical if they exist in the tensile region of the toughened glass, and are large enough to generate cracks. Critical inclusions tend to be between 40 and 250 mm, which is too small to be obvious on visual inspection, although large enough to see with the naked eye once located.
The unexpected breakage of toughened glass can be inconvenient and costly, alarming, and sometimes dangerous, so a great deal of effort has been dedicated to preventing it. The most effective method has been found to be ‘heat soaking’, in which the toughened glass is re-heated to a temperature at which critical nickel sulphide inclusions will undergo the transition to the beta phase and cause breakage then and there. Heat soaking is defined in the European standard for heat-soaked thermally toughened soda lime silicate glass BS EN 14179, and involves raising the glass to a temperature of between 280°C and 300°C for two hours.
Heat Strengthening
The additional strength of toughened glass is valuable for providing resistance to wind pressure and thermal stress, but its fracture pattern makes it prone to collapse once broken and this can be a safety issue if it falls from a tall building.
Heat-strengthened glass has about twice the strength of annealed (float) glass and enough thermal shock resistance to suit any practical building example, but its residual compressive stress is low enough not to break the pane into multiple fragments. The intention is that heatstrengthened glass breaks more or less like annealed glass, with a radiating pattern of cracks and very little branching, so that all the fragments extend to the perimeter and can be retained by the glazing system.
The European standard for heat-strengthened glass (EN1863) defines the required fracture pattern and sets a minimum characteristic bending strength. Heat strengthening is carried out with the same machinery as toughening, and the process steps are the same, with the exception of the quenching stage, and the glass is cooled more slowly so that the temperature difference between the core and the surfaces is carefully controlled.
Heat-strengthened glass is not a safety glass because when it breaks it does not break in a safe manner that would avoid injury to someone impacting it. The combination of higher strength, thermal shock resistance and radial breakage pattern makes heatstrengthened glass very useful when laminated with other panes for structural applications.
Chemical Strengthening
Chemical strengthening is an alternative way of establishing compressive stress in the surface of the glass without introducing distortion, and which can be applied to any thickness of glass. The process is, in essence, one of replacing sodium ions (from the soda flux) at the surface of the glass with potassium ions, which are bigger. The wedging action caused by the substitution generates a compressive stress in a very shallow layer of the surface, which is balanced by a very low tensile stress in the core. It is carried out by placing the glass in a bath of hot potassium chloride, the temperature, concentration and duration of immersion being proprietary parameters.
Despite its cost and other limitations, chemical strengthening is the only option for some shaped glasses, such as conics, and is widely used for the layers of laminated aircraft windows, where highstrength thin glass is required for weight saving. Very little strain energy is stored in the panel by the chemical strengthening process, so the treated glass does not fragment like toughened glass, and it is not a safety glazing material unless laminated.
FORMING PROCESSES
Bending
Flat glass can be bent by heating to around 700°C and allowing to slump into or over a mould, often made of steel sheet or tubes draped with refractory fabric. Such moulds can be relatively inexpensive and are suitable for ruled surfaces, especially cylindrical or sinusoidal forms. If a laminated panel is required, a pair of blanks or up to four to make an insulating unit, are stacked together, separated with a mineral powder to prevent adhesion when the glass is hot.
The blank is then placed on the mould and the kiln closed and fired. The glass softens and sags into or slumps over the mould and the temperature is lowered to the annealing range, through which it is lowered slowly to allow the stresses of bending to relax and to prevent the creation of residual stress. Double curves such as segments of a sphere or ellipsoid, which require stretching, are often approximated by sag bending on a ‘skeleton mould’.
This consists of a shaped steel rail defining the required perimeter profile of the glass, supported by a welded lightweight steel framework, with a reference point indicating the maximum depth of curvature of the required form (Fig. 5).

An over-sized flat blank of glass is placed on the high points of the skeleton mould, and the kiln heated to the bending temperature. While the glass temperature rises, it has to be observed as it softens and deflects, and the kiln temperature quickly dropped to arrest its flow when the glass reaches the desired depth of curvature.
Great skill and experience are required in the design of the mould and the application of heat to the glass, to influence how and where it flows to get the best approximation of the intended form. The kiln bending process is adapted to create a wide variety of unique textures and patterns in a process known as ‘kiln forming’ or ‘casting’.
Here the flat glass blank is placed on a full surface mould of ceramic or steel and heated until it conforms to the surface texture of the mould.
Bending and Tempering
Curved or bent toughened glass cannot be made by the processes described above, because both surfaces have to be exposed to cooling air in order to achieve the required residual stress. Special tempering machines are used, in which the rollers of the quenching section are mounted on numerically-controlled mechanisms, and in some cases are segmented and flexible.
When the hot glass enters the quenching section, the rollers are moved to the programmed shape and continue to oscillate the glass as it bends under its own weight, before air is blown from above and below to temper the glass. If laminated bent toughened glass is required, each sheet of glass has to be processed separately and with sufficient consistency that pairs can be laminated together.
There are no standards for the quality of curved glass at present, so designers have to specify the requirements for individual projects in considerable detail.
Channel Glass
In an adaptation of the rolled plate process, a rigid glass channel can be created, which has much greater strength and stiffness in one direction than a sheet of glass. Longitudinal wires can also be introduced into the glass to provide a very limited degree of stabilisation after breakage. Glass channels have been widely used as an inexpensive means of providing daylight, especially for industrial buildings, but more recently for architectural applications, because they can span between floors without the need for framing.
Decoration Processes
Sand Blasting
A simple way to modify the transparency of glass is to sand blast one surface to create a texture that scatters the light and diffuses the image seen through the pane. A range of textures can be produced and the surface can be sculpted to achieve surface relief.
Automated sand blasting is used to achieve consistent texture, and can be combined with masking to apply patterns and graphic designs, while manual blasting allows more creative effects. Abrasive granules are fired at the surface of the glass by a stream of compressed air and create a mass of pits and tiny cracks, which act to reflect and refract light in random directions. The surface tends to absorb grease and oils readily, so it shows finger marks and is difficult to clean.
Therefore a sand-blasted surface is commonly sealed with a proprietary dirt-repellent treatment before delivery. Sand-blasted surfaces provide higher friction than smooth glass surfaces when wet, so are sometimes used to reduce the risk of slipping on glass flooring.
Acid Etching
Glass is resistant to most chemicals but can be dissolved by hydrofluoric acid. The areas not to be etched are masked and the glass set up so that the surface can be flooded with hydrofluoric acid. Variations of the solution and the etching time are used to create a subtle range of textures and, being a skilled craft, acid etching is a costly process.
Acid-etched glass diffuses light rather less than sand-blasted because the surface is more undulating and granular, lacking the numerous tiny fracture faces that characterise the sand-blasted finish. However, acid-etched glass is easier to clean and does not mark so readily.
Fritting
Ceramic ‘frit’ is a mixture of low melting-point clear glass and mineral pigments in the form of a water-based paste, which can be applied to glass, dried and then fired to create permanent enamel. A wide range of colours is available to special order but most processors offer a limited range of standards, usually including black and white, a few muted colours and a translucent white ‘mist frit’ that very effectively mimics sand-blasted or acid-etched glass.
Abrasive granules can be added to frit to provide a non-slip surface. The frit is fired during the heating phase of the toughening or bending process, and the vast majority of fritted glass is toughened or heat strengthened. Patterns such as dots, meshes and lines, are usually applied by ‘silk-screen printing’, in which the frit is forced through a masked screen by a squeegee.
Recently, a digital process has been devised by means of which masking of the screen can be carried out, which allows images to be incorporated easily, but the cost of screens remains significant. Some processors are able to apply several layers of different colour frit by successive printing steps. Frit can also be applied directly to the glass by the ‘ink-jet’ process on a scale of up to 3 m by 4 m. Direct digital printing in this way can reproduce digital artwork in colour but is currently a slow process, and therefore more suited to individual designs rather than repetitive patterns.
Stained Glass
The ancient technique of ‘stained glass’, in which pigments were fired directly into the surface of clear glass, has been updated recently. Instead of ink-jet printing with a liquid frit, this process deposits dry powder pigments onto the glass under digital control. When the glass is fired, the pigments flow and merge, which results in less definition than a digitally printed frit but much higher translucency and blending of colour.
Printing
When glass is laminated, the plastic interlayer material can be digitally printed with patterns or images in full colour. The resolution of printing onto plastics using organic inks is currently better and more flexible than directly printing frit onto the glass.
LAMINATING
The brittleness of glass itself cannot be modified, but lamination provides a means of producing a glass pane with a form of ductility. Lamination involves sandwiching a layer of ductile plastic between layers of glass, and can be used to make products with a range of performance, from a modest level of protection in the case of human impact, to the ability to stop high velocity ballistics.
Most glass is laminated by the ‘nip roller and autoclave’ process, using a polyvinyl-butyral (PVB) clear interlayer. The glass is usually in the annealed form, but can be toughened or heat strengthened and may be coated or decorated before lamination. A sheet of PVB is laid onto one pane and another placed on top, in a ‘clean room’ section of the factory where the atmosphere is controlled and the potential for contamination minimised (Fig. 6).
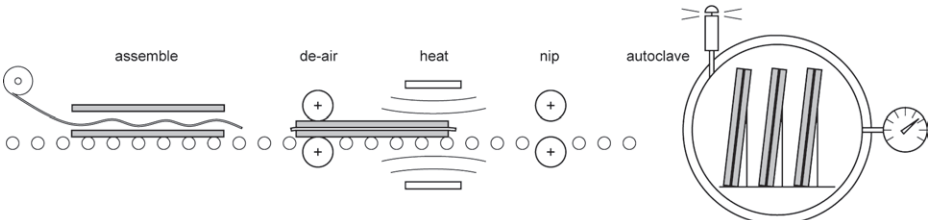
The pane passes through a pair of rubber-coated rollers, which squeeze out most of the air trapped between the glass and the textured surface of the PVB. Once the sandwich has been heated and passed through another pair of ‘nip rollers’, the glass is stacked on edge upon a ‘stillage’ and loaded into an autoclave, where the temperature is raised to around 130°C and the pressure is raised to around 16 bar (16 atmospheres or around 1600 kPa).
The small amount of trapped air dissolves in the hot PVB interlayer, which wets the surface completely. The temperature and pressure are reduced in a controlled way and the glass is removed from the autoclave. Glass that is curved, deeply textured or very thick, is not suitable for the nip roller process and a vacuum bag is used in its place.
This is a flat bag, formed around the assembled glass from sheets of polyester film and sealed at the perimeter, from which the air is evacuated through a valve. PVB is the most widely used interlayer because it is reasonably economical, extremely ductile and energy absorbing and resistant to ultraviolet light. One of the alternatives to PVB is ethylene-vinyl acetate (EVA), which is also supplied in sheet form on a roll, and is widely used in the lamination of photo-voltaic cells into modules encapsulated between panes of glass.
Panes laminated with EVA tend to be more resistant to high temperatures and less affected by moisture ingress at the edges, although EVA is not as ductile and tough as PVB. Polyurethane (PU) sheet interlayers have good heat resistance, remaining rubbery and elastic over a wide range of temperatures, and bond well to sheet plastics like polycarbonate. This makes them a preferred choice for some security glazing applications against ballistics and manual attack.
The clarity of polyurethane interlayers is good but the cost is higher than for other interlayers. The latest sheet interlayer to have a significant impact on architectural glazing is only available from one supplier, but has been adopted by a number of processors to offer different mechanical properties from PVB.
DuPont Sentry Glass has an ‘ionomer’ interlayer; developed from a class of plastics originally used for the skin of golf balls, it has high strength and stiffness, which it retains up to around 50°C. Within the polymer structure are ionic bonds that are mobile at processing temperatures but prevent the polymer chains from sliding over each other at service temperatures.
Ionomer interlayer bonds strongly to glass and very strongly to metals. The principal advantage of ionomer interlayer is its high shear modulus and resistance to stress relaxation, which allows designers to take advantage of composite action between panes of glass when laminated together, providing the service temperature is moderate.
A number of alternative laminating materials are also available, collectively known as ‘cast in place’ (CIP), ‘cold pour’ or ‘resin’ laminates. These all have the advantage for the processor of being usable with very little investment in equipment. Cast in place interlayers may be chemically cured, such as two-part acrylic (PMMA) or two-part acrylate, polymetyl methacrylate, or single-part resins cured by ultra-violet light (UV), which are for the most part acrylic/acrylate.
Additional layers of material can be incorporated into the laminating process, to enhance technical performance or provide decoration. Multi-layer drawn polyester films provide a dimensionally stable substrate for coating and printing, which can be laminated between glass using two layers of PVB. Decorative materials like fine fabrics, thin wood veneers, metal mesh, expanded metal and even leaves, have also been laminated between glasses, generally by the vacuum bag process.
INSULATING UNIT MANUFACTURE
Solid glass is a poor conductor of heat compared to metals, but not an adequate insulator for the level of thermal performance required of modern buildings. The addition of layers of plastics by laminating does not enhance the thermal performance to any useful degree, so the majority of glass currently used in building façades is in the form of insulating glazing units (IGU), either double- or triple-glazed.
The idea of an IGU is to trap a layer of dry air or gas between panes of glass and permanently seal the perimeter to prevent the gas escaping and to prevent moisture penetrating into the dry cavity. It is important to keep the gas in the cavity dry because the outer pane of glass will reach low temperatures during cold weather and condensation would form on its inside face unless the moisture had been removed.
The width of the cavity should be sufficient to provide an insulating layer of still air or gas, but not so wide that convection currents can become established, which would reduce the insulation effect by transferring heat between the panes.
The spacer between the panes contains a desiccant material to absorb moisture from the air trapped in the cavity when the unit is assembled. Desiccants were originally silica gel but are now ‘molecular sieve’ materials that can absorb large quantities of moisture and lower the dew point inside the unit to around -80°C when first sealed. The low humidity within a unit creates a large vapour pressure difference between outside and inside the cavity, which drives moisture past or through the seal materials over time.
The primary vapour seal between the metal spacer and the glass is a bead of poly-isobutylene (PIB) in a good quality unit because PIB has very low vapour permeability and remains sticky and flexible over a wide range of temperatures.
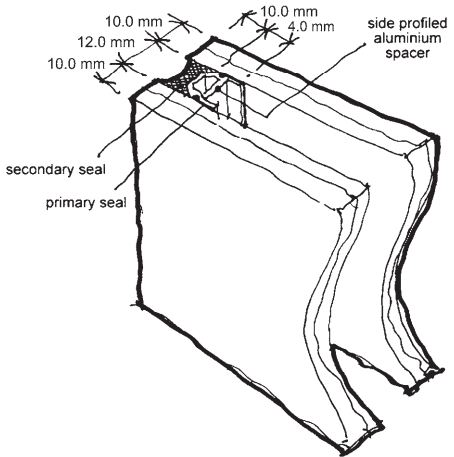
The bead of PIB, also known simply as ‘butyl’ has to be continuous and to close any junctions in the spacer to create a complete seal (Fig. 7). Changes in the relative pressure between the inside and outside of the unit tend to force the glass away from the butyl primary seal on the spacer, so a ‘secondary sealant’ is used to hold the two panes together and locate the spacer.
Polysulphide secondary sealants are popular for units that will be framed so that the polysulphide will not be exposed to UV radiation, which breaks down its adhesion to glass, and some polyurethane sealants are used for the same purpose. Units whose edges will be exposed to sunlight, for frameless glazing or structural silicone glazing, for example, are sealed with silicone secondary sealants, which are resistant to UV but often tend to be more flexible.
Extruded aluminium spacers are increasingly being replaced with folded stainless steel, pultruded fibre-reinforced plastics or polymer foam spacers, which have reduced thermal conductivity. These types are collectively known as ‘warm-edge’ spacers because they reduce the cold-edge effect that results from using a conductive metal spacer, and they have a range of thermal performance and a variety of individual advantages.
FIRE-RESISTING GLASSES
The process of making wired glass was described above, and it is still commonly used for smaller panes where integrity against fire is required and appearance is not paramount. Non-wired fire-resisting glasses fall broadly into three performance groups and two different technology groups.
The performance of fire-resisting glass is classified according to BS EN 357 in terms of ‘integrity only’ (E), ‘integrity with radiation protection’ (EW) and ‘integrity with insulation’ (EI). If a glass prevents the passage of smoke and flame for a specified period under test conditions then it can be classified as providing ‘integrity’ for the given period – 30 minutes, 60 minutes or 120 minutes, for example. Higher-performing ‘integrity and insulation’ glasses have an insulating effect and prevent the non-fire side of the glass rising to dangerous temperatures that would prevent people passing by the outside during a fire.
Bororsilicate glass is successful at providing ‘integrity only’ protection, because it has a lower coefficient of thermal expansion than soda lime glass, so it is able to resist more severe thermal shock and it is usually toughened, which further increases its thermal shock resistance.
Toughened borosilicate has been successfully fire tested in certain sizes up to 60 minutes when framed on top and bottom edges only, with special sealant in the butt joint between panes. Some laminated soda lime glass products incorporating mineral based interlayers are able to provide 60 or 120 minutes integrity when the interlayer foams to provide the second layer of glass with enough protection to control thermal shock.
There are also some fire-resistant products that are essentially highly toughened soda lime glass with smooth edge work in narrow frames that maximise the strength of the glass and minimise the thermal shock it experiences during the heating phase of a fire test. Multiple layers of annealed low-iron glass, laminated with a transparent layer of hydrated salts, can provide a combination of integrity and insulation.
When exposed to fire, the hydrated salt interlayer turns into a foam (intumesces) and expands as the glass softens. The foamed interlayer insulates subsequent layers of glass and blocks the transfer of radiant heat, so that the non-fire side of the glass does not present a burn hazard. This kind of glass can be used to protect an escape route past a window or glass screen because the foamed interlayer prevents the passage of heat and masks the fire from view.
Other products consisting of two layers of glass enclosing a thick intumescent gel provide similar performance. Hardwood frames with deep rebates or steel frames covered in thermal insulation are the preferred forms of fire framing, and the combination of glass and framing is crucial at any particular size of panel and duration of fire resistance.