Alloying of Metals
Approximately 80% of the one-hundred-plus elements in the periodic table can be classed as metals. A number of these possess combinations of availability and properties that lead to their use as engineering metals where mechanical strength is needed. The most widely used engineering metal is iron, which is the main constituent of the iron-based alloys termed steels. Some other structural metals that are widely used are aluminum, copper, titanium, magnesium, nickel, and cobalt.
Additional common metals, such as zinc, lead, tin, and silver, are used where the stresses are quite low, as in various low-strength cast parts and solder joints. The refractory metals, notably molybdenum, niobium, tantalum, tungsten, and zirconium, have melting temperatures somewhat or even substantially above that of iron (1538◦C). Relatively small quantities of these are used as engineering metals for specialized applications, particularly where high strength is needed at a very high temperature. Some properties and uses for selected engineering metals are given in Table 1.
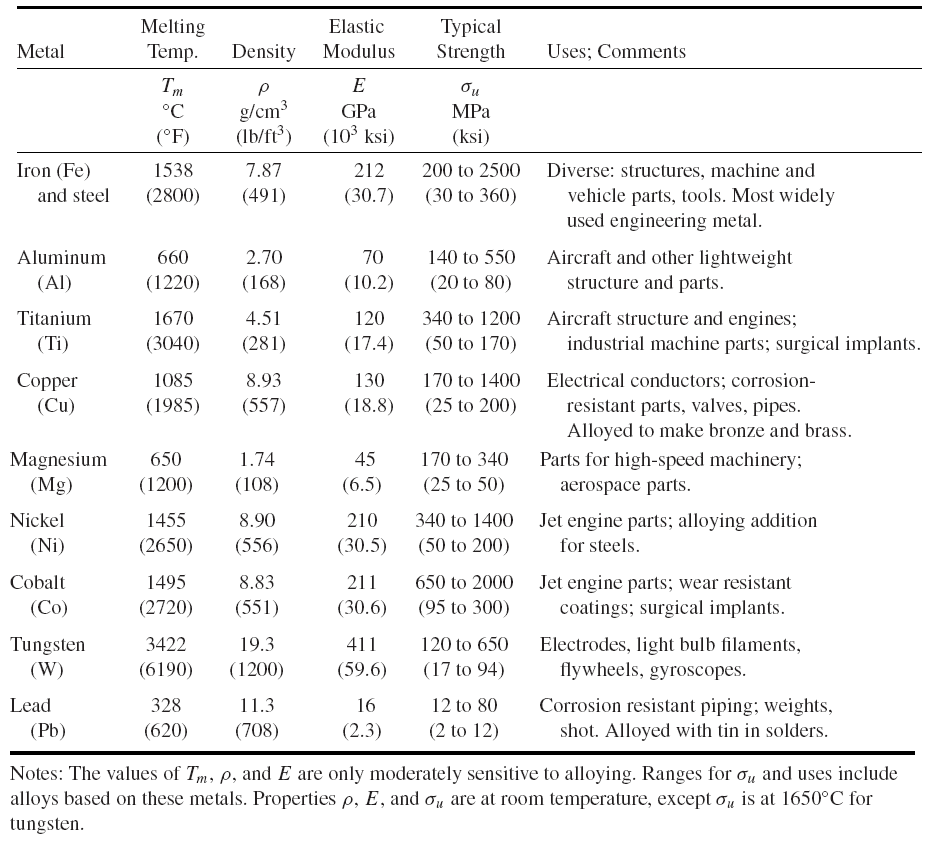
Alloying and Processing of Metals
A metal alloy is usually a melted-together combination of two or more chemical elements, where the bulk of the material consists of one or more metals. A wide variety of metallic and nonmetallic chemical elements are used in alloying the principal engineering metals. Some of the more common ones are boron, carbon, magnesium, silicon, vanadium, chromium, manganese, nickel, copper, zinc, molybdenum, and tin.
The amounts and combinations of alloying elements used with various metals have major effects on their strength, ductility, temperature resistance, corrosion resistance, and other properties.
For a given alloy composition, the properties are further affected by the particular processing used. Processing includes heat treatment, deformation, and casting. In heat treatment, a metal or alloy is subjected to a particular schedule of heating, holding at temperature, and cooling that causes desirable physical or chemical changes. Deformation is the process of forcing a piece of material to change its thickness or shape. Some of the means of doing so are forging, rolling, extruding, and drawing, as illustrated in Fig. 1.
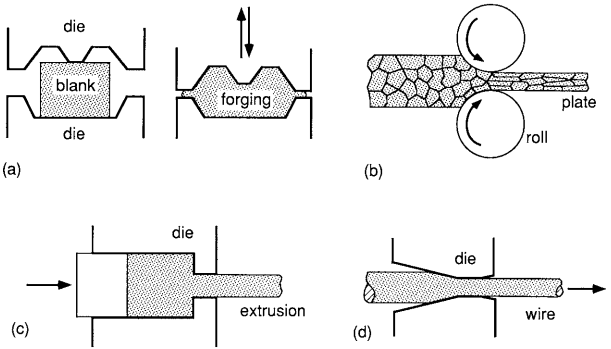
Casting is simply the pouring of melted metal into a mold so that it conforms to the shape of the mold when it solidifies. Heat treatment and deformation or casting may be used in combination, and particular alloying elements are often added because they influence such processing in a desirable way. Metals that are subjected to deformation as the final processing step are termed wrought metals to distinguish them as a group from cast metals.
The details of alloying and processing are chosen so that the material has appropriate temperature resistance, corrosion resistance, strength, ductility, and other required characteristics for its intended use. Recalling that plastic deformation is due to the motion of dislocations, the yield strength of a metal or alloy can usually be increased by introducing obstacles to dislocation motion.
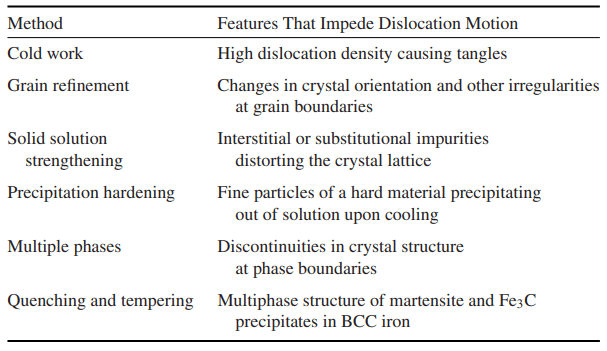
Such obstacles can be tangles of dislocations, grain boundaries, distorted crystal structure due to impurity atoms, or small particles dispersed in the crystal structure. Some of the principal processing methods used for strengthening metals are listed, along with the type of obstacle, in Table 2. We will now discuss each of these methods.
Cold Work and Annealing
Cold work is the severe deforming of a metal at ambient temperature, often by rolling or drawing. This causes a dense array of dislocations and disorders the crystal structure, resulting in an increase in yield strength and a decrease in ductility.
Strengthening occurs because the large number of dislocations form dense tangles that act as obstacles to further deformation. Hence, controlled amounts of cold work can be used to vary the properties. For example, this is done for copper and its alloys.
The effects of cold work can be partially or completely reversed by heating the metal to such a high temperature that new crystals form within the solid material, a process called annealing. If this is done following severe cold work, the recrystallized grains are at first quite small. Cooling the material at this stage creates a situation where strengthening is said to be due to grain refinement, because the grain boundaries impede dislocation motion.
A long annealing time, or annealing at a higher temperature, causes the grains to coalesce into larger sizes, resulting in a loss of strength, but a gain in ductility. The microstructural changes involved in cold working and annealing are illustrated in Fig. 2.
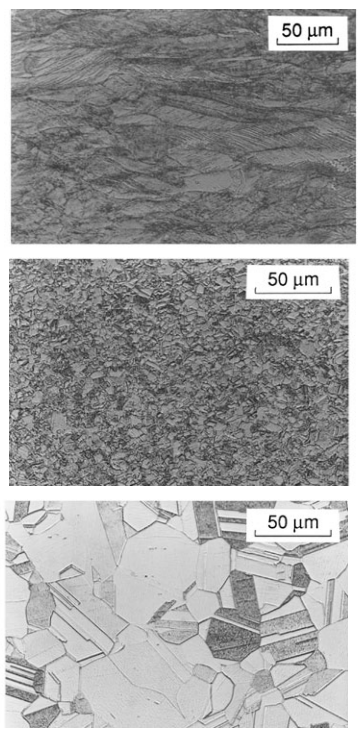
annealed one hour at 375◦C (middle); and annealed one hour at 500◦C (bottom).
Solid Solution Strengthening
Solid solution strengthening occurs as a result of impurity atoms distorting the crystal lattice and thus making dislocation motion more difficult. Note that alloying elements are said to form a solid solution with the major constituent if their atoms are incorporated into the crystal structure in an orderly manner. The atoms providing the strengthening may be located at either interstitial or substitutional lattice positions.
Atoms of much smaller size than those of the major constituent usually form interstitial alloys, as for hydrogen, boron, carbon, nitrogen, and oxygen in metals. Substitutional alloys may be formed by combinations of two or more metals, especially if the atomic sizes are similar and the preferred crystal structures are the same.
As might be expected, the effect of a substitutional impurity is greater if the atomic size differs more from that of the major constituent. This is illustrated by the effects of various percentages of alloying elements in copper in Fig. 3.
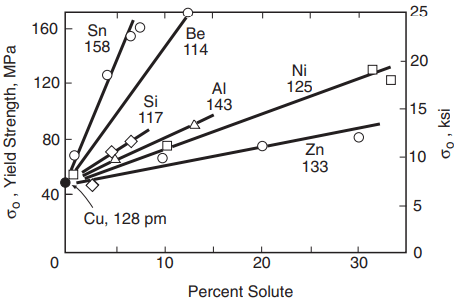
Zinc and nickel have atomic sizes that do not differ very much from that of copper, so that the strengthening effect is small. But the small atoms of beryllium and the large ones of tin have a dramatic effect.
Precipitation Hardening and Other Multiple Phase Effects
The solubility of a particular impurity species in a given metal may be quite limited if the two elements have dissimilar chemical and physical properties, but this limited solubility usually increases with temperature. Such a situation may provide an opportunity for strengthening due to precipitation hardening.
Consider an impurity that exists as a solid solution while the metal is held at a relatively high temperature, but also assume that the amount present exceeds the solubility limit for room temperature. Upon cooling, the impurity tends to precipitate out of solution, sometimes forming a chemical compound in the process.
The precipitate is said to constitute a second phase as the chemical composition differs from that of the surrounding material. The yield strength may be increased substantially if the second phase has a hard crystal structure that resists deformation, and particularly if it exists as very small particles that are distributed fairly uniformly.
Also, it is desirable for the precipitate particles to be coherent with the parent metal, meaning that the crystal planes are continuous across the precipitate particle boundary. This causes distortion of the crystal structure of the parent metal over some distance beyond the particle, enhancing its effect in making dislocation motion more difficult.
For example, aluminum with around 4% copper forms strengthening precipitates of the intermetallic compound CuAl2. The means of achieving this is illustrated in Fig. 4. Slow cooling allows the impurity atoms to move relatively long distances, and the precipitate forms along the grain boundaries where it has little benefit.
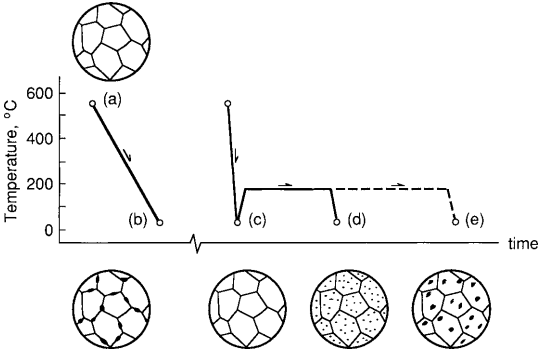
However, substantial benefit can be achieved by rapid cooling to form a supersaturated solution and then reheating to an intermediate temperature for a limited time. The reduced movement of the impurities at the intermediate temperature causes the precipitate to form as fairly uniformly scattered small particles.
However, if the intermediate temperature is too high, or the holding time too long, the particles coalesce into larger ones and some of the benefit is lost, the particles being too far apart to effectively impede dislocation motion. Thus, for a given temperature, there is a precipitation (aging) time that gives the maximum effect. The resulting trends in strength are illustrated for a commercial aluminum alloy, where similar precipitation hardening occurs, in Fig. 5.
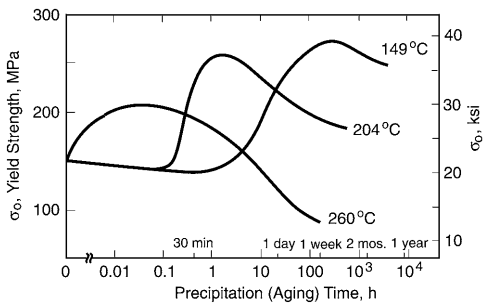
If an alloy contains interspersed regions of more than one chemical composition, as for precipitate particles, as just discussed, a multiple phase situation is said to exist. Other multiple phase situations involve needlelike or layered microstructural features, or crystal grains of more than one type.
For example, some titanium alloys have a two-phase structure involving grains of both alpha (HCP) and beta (BCC) crystal structures. Multiple phases increase strength, because the discontinuities in the crystal structure at the phase boundaries make dislocation motion more difficult, and also because one phase may be resistant to deformation.
The twophase (alpha–beta) structure just mentioned for titanium provides a portion of the strengthening for the highest strength titanium alloys. Also, the processing of steels by quenching and tempering, which will be discussed in the next article, owes its benefits to multiple phase effects.
Thanks for reading of “alloying and processing of metals.”